Table of Contents
Preface of Respiratory Chain:
The respiratory or electron transport (ETC) chain is important to cellular respiration. This essential mechanism enables cells to derive energy from nutrients, converting it into ATP, the cellular energy currency. Various environmental conditions, including salinity—the quantity of dissolved salts in the surrounding medium—can affect the efficacy of the electron transport chain. Although organisms have adapted to various salinities, low salinity poses distinct hurdles to cellular activities, particularly the intricate electron transfer pathways facilitating ATP generation.
Understanding electron transfer mechanisms in the respiratory chain under low salinity is critical for fundamental biological insights and applications in biotechnology, aquaculture, and environmental science. This article investigates the Respiratory Chain, the influence of low salinity on the electron transport chain, its consequent effect on ATP synthesis, and the extraordinary adaptations that organisms have evolved to address these challenges. Schematic view of electron and proton transfer by CIII2 and CIV.
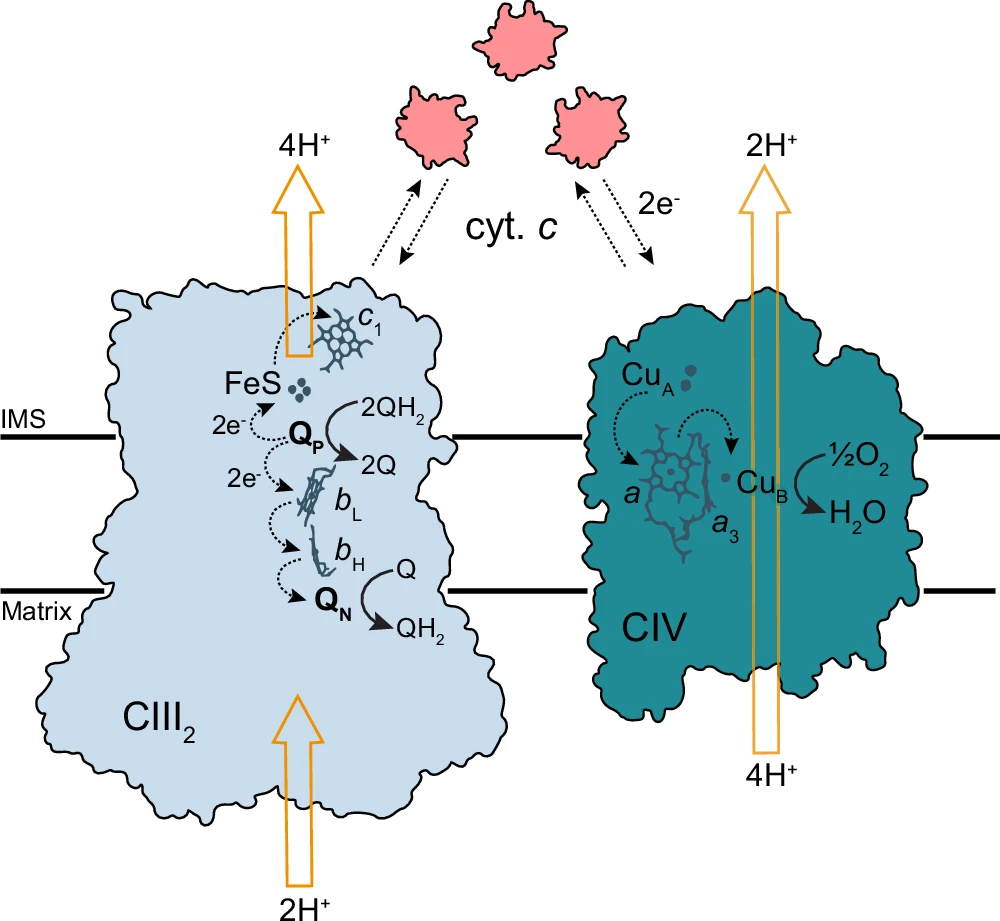
The Fundamentals of the Respiratory Chain:
The respiratory chain is made up of a series of protein complexes that are located inside the inner mitochondrial membrane (or plasma membrane in prokaryotes). This chain facilitates the transport of electrons from donors such as NADH and FADH2 to oxygen, the ultimate electron acceptor. It generates a proton gradient that facilitates ATP synthesis.
Essential Elements of the Respiratory Chain:
The respiratory chain consists of four primary complexes:
Complex I (NADH dehydrogenase): Complex I, which is NADH dehydrogenase, starts the process by taking electrons from NADH, moving them to ubiquinone (coenzyme Q), and pushing protons across the membrane.
Complex II (succinate dehydrogenase): Helps electrons move from FADH2 to ubiquinone without moving protons, but it still has a part to play in the electron transfer process.
Complex III (Cytochrome bc1 complex): Complex III, also known as the Cytochrome BC1 complex, takes electrons from ubiquinone and sends them to cytochrome c. It also helps move protons around to keep the gradient going.
Complex IV (Cytochrome c oxidase): Catalyzes terminal electron transfer to oxygen, reduces it to water and aids in proton translocation.
Mobile electron carriers, such as ubiquinone and cytochrome c, transport electrons between these complexes, facilitating an uninterrupted flow down the chain. Each stage releases energy that transports protons from the mitochondrial matrix to the intermembrane space, establishing the proton gradient essential for ATP production by ATP synthase.
Electron Transfer: Mechanism of Action
The disparities in redox potential between electron donors and acceptors propel the meticulously orchestrated process of electron transfer. NADH and FADH2, produced by metabolic pathways such as glycolysis and the citric acid cycle, transfer electrons to the chain. Electrons release energy as they traverse the protein complexes in the chain.
The mitochondrial membrane uses this energy to translocate protons, resulting in an electrochemical gradient known as the proton-motive force. The buildup of protons in the intermembrane gap establishes a substantial concentration gradient relative to the mitochondrial matrix. Protons re-enter the matrix via ATP synthase, which harnesses this movement to synthesize ATP from ADP and inorganic phosphate.
Complex IV is the terminal phase of electron transfer, where electrons transfer to oxygen, which then combines with protons to generate water. This stage is essential for sustaining electron flow; without oxygen, the entire process ceases, resulting in diminished ATP generation and energy deficiency. Activity as a function of cyt. c concentration.
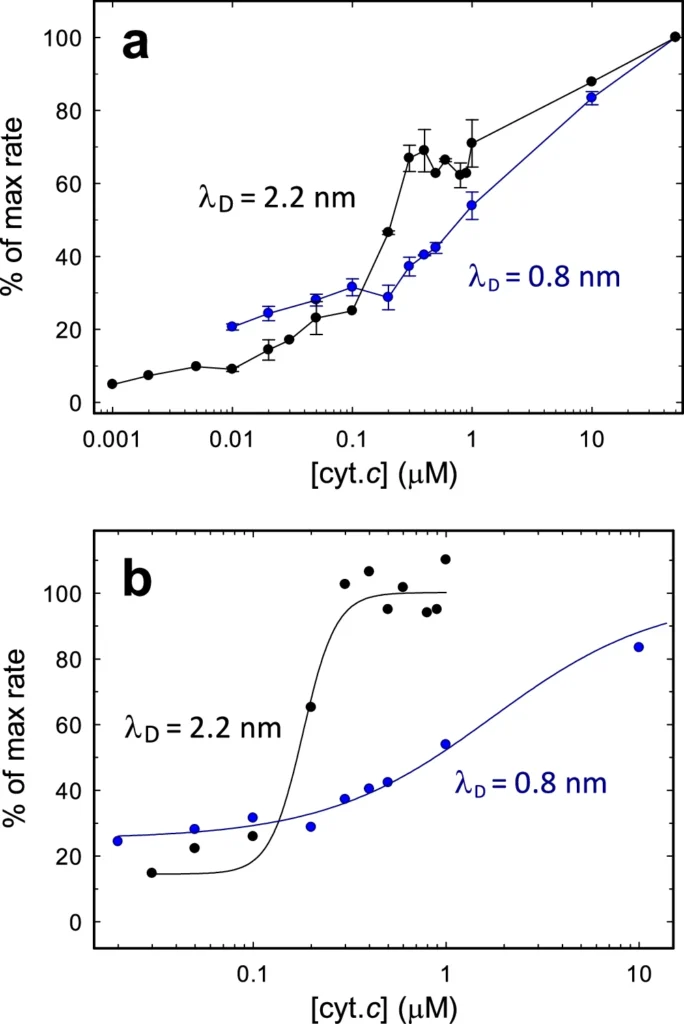
Salinity: An Essential Environmental Parameter
Salinity quantifies the concentration of dissolved salts in water, mostly consisting of ions such as sodium (Na+), potassium (K+), calcium (Ca2+), chloride (Cl-), and magnesium (Mg2+). Natural settings display significant differences in salinity, from freshwater lakes and rivers with minimal salinity to hypersaline habitats such as salt flats.
Organisms have heightened sensitivity to salinity variations because their cellular activities rely on a precise ion balance. This equilibrium is essential for sustaining osmotic pressure, membrane potential, and the functionality of proteins and enzymes, especially those engaged in electron transport and ATP synthesis.
Natural ecosystems with diverse salinity levels:
Marine environments: Oceans possess an average salinity of 35 parts per thousand (ppt), although this may fluctuate based on geographical location and depth. Organisms living in marine environments need to adapt to the elevated salinity conditions.
Freshwater environments: Freshwater ecosystems, including rivers, lakes, and ponds, have significantly lower salinity levels, typically below 0.5 ppt. Freshwater species encounter distinct problems relative to marine creatures, especially in regulating internal ion equilibrium.
Estuaries and brackish water: These transitional areas between freshwater and saltwater exhibit variable salinity levels, necessitating that organisms adapt to continual changes in their external environment.
Normal Salinity’s Impact on the Respiratory Chain:
Under standard salinity levels, electron transport in the Respiratory Chain occurs effectively. The ionic equilibrium within and around the cell, particularly in the mitochondria, facilitates the optimal operation of the proton pumps in the respiratory chain. Sodium, potassium, and chloride ions are essential for sustaining membrane potential, which aids in proton pumping and the establishment of the proton gradient.
When the environment is stable and the salt level is normal, cells can control the flow of ions across their membranes. This makes electron transfer, proton pumping, and ATP production easy. The proton-motive force is robust, allowing ATP synthase to generate ATP consistently, which is essential for cellular energy requirements.
The Effect of Reduced Salinity on Cellular Function:
Cells exposed to low salinity conditions can cause a variety of disturbances. The equilibrium of ions, especially Na+ and K+, is essential for sustaining membrane potential and cellular homeostasis. Reduced salinity may result in water entering the cell due to osmotic pressure, leading to cellular swelling and possible damage to structures, including membranes.
Electrolyte Discrepancy:
Low salinity settings can impair the ion gradients necessary for the electron transport chain’s operation. Sodium and potassium ions are essential for preserving the electrochemical gradient across the mitochondrial membrane. Low salinity results in diminished availability of these ions, potentially undermining the membrane potential and the cell’s capacity to efficiently pump protons.
Osmotic Stress:
Reduced salinity elevates osmotic pressure, compelling water to enter the cell. This could lead to mitochondrial enlargement and disruption of the inner membrane, where the respiratory chain resides. Alterations to the inner membrane’s structure can adversely impact the organization and functionality of the protein complexes responsible for electron transport. Concentration and fraction reduced cyt. c.
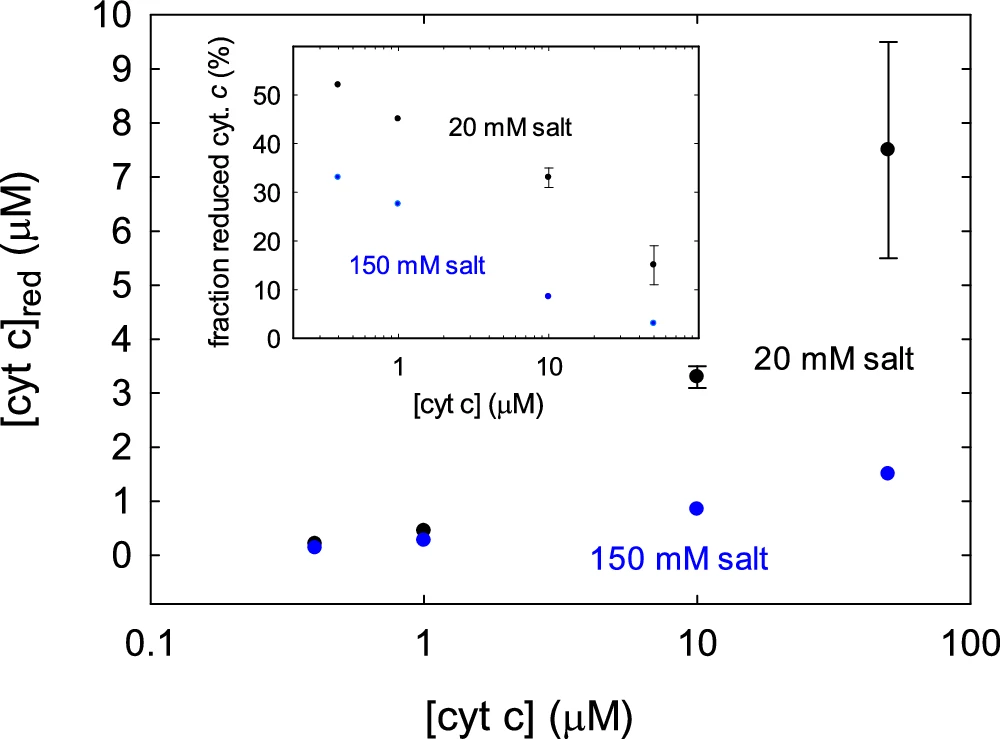
Alterations in Electron Transfer Mechanisms with Reduced Salinity:
Disturbances in the proton-motive force frequently reduce the efficacy of electron transport under low-salinity conditions. The proton gradient across the mitochondrial membrane is essential for ATP synthesis, and its disturbance can lead to significant repercussions.
Efficiency of Proton Pumping:
Ion imbalances caused by low salinity can disrupt the regular function of proton pumps. Complexes I, III, and IV depend on a meticulously controlled ion flow to translocate protons across the membrane. In the absence of a steady ionic environment, these proton pumps may operate at diminished efficiency, leading to a compromised proton gradient. This results in diminished ATP generation, as a reduced number of protons are available to propel ATP synthase.
Decreased electron transfer rate:
Reduced salinity can impede the rate of electron transport among complexes. This may be due to structural changes in the protein complexes or modified interactions between electron carriers such as ubiquinone and cytochrome c. As a result, oxygen receives fewer electrons, which lowers the overall rate of ATP synthesis.
Proton-Motive Force and ATP Synthesis in Low Salinity:
Low salinity settings frequently diminish the proton-motive force, which serves as the impetus for ATP production. Active transport of protons across the mitochondrial membrane creates the proton gradient, and any interference with this mechanism results in diminished ATP synthesis.
Implications of Diminished Proton GradientP:
When the proton-motive force goes down, ATP synthase can’t bring as many protons into the mitochondria, which directly affects the rate of ATP synthesis. This energy shortfall can impede a cell’s capacity to perform vital processes, such as growth, repair, and metabolism.
In severe instances, cells may undergo energy depletion, potentially instigating apoptosis (programmed cell death) or necrosis (uncontrolled cell death). The capacity to acclimatize to these circumstances is essential for survival.
The stability of proteins in the respiratory chain at low salinity levels is a concern:
Some proteins in the electron transport chain, like Complex I (NADH dehydrogenase) and Complex IV (cytochrome c oxidase), are very sensitive to changes in their environment. At low salinity, these proteins may destabilise, resulting in diminished functionality.
Salinity induces protein stress:
Reduced salinity may cause respiratory chain proteins to denatur or misfold. These proteins depend on particular ionic conditions to preserve their three-dimensional conformation, essential for their catalytic activity. Ionic imbalance can happen in low-salinity conditions, which can make proteins lose their functional shape. This makes them less able to facilitate electron transfer.
Mechanisms for Stabilising Adaptive Proteins:
Certain species have developed ways to safeguard their proteins against the destabilising impacts of low salinity. As part of these changes, the body makes the right solutes, like proline or trehalose, which help keep protein structure stable and stop it from breaking down under osmotic stress.
Organisms’ Adaptations to Low Salinity:
Notwithstanding the difficulties presented by low salinity, numerous organisms have developed extraordinary adaptations that enable them to sustain electron transfer efficiency and ATP synthesis. These adaptations may be physiological, biochemical, or behavioural, contingent upon the organism and its environment.
Mechanisms of Ion Transport:
Certain species have specialized ion transporters and pumps that help maintain intracellular ion equilibrium, even in low salinity conditions. These transporters actively facilitate the movement of sodium and potassium ions across the cell membrane, hence maintaining the electrochemical gradient essential for electron transfer.
Compatible Solutes:
Some organisms synthesise organic compounds, referred to as compatible solutes, to mitigate osmotic stress. These compounds, such as proline, glycine betaine, and trehalose, contribute to the stabilization of cellular structures, including proteins and membranes, despite salt variations. These solutes are essential for sustaining protein activity, hence enhancing electron transfer efficiency in low-salinity settings.
Membrane Modifications:
Certain organisms can modify the lipid composition of their membranes in reaction to low salinity. These modifications preserve membrane fluidity and integrity, which are crucial for the optimal operation of the respiratory chain. Organisms can prevent membrane damage and maintain proton pumping and ATP synthesis by modifying the ratio of saturated to unsaturated fatty acids in their membranes.
Marine and Freshwater Organisms: Comparative Analyses
Marine and freshwater organisms have different problems with salt. Freshwater organisms must manage low salinity, while marine species typically adapt to high-salinity habitats. Both categories of species have developed distinct techniques to maintain the functionality of their respiratory chains within their specific environments.
Marine Organisms:
Fish and invertebrates, among other marine creatures, adapt to elevated salt levels. Nevertheless, certain species, such as salmon, are required to undergo a transition between saline and freshwater habitats throughout their life cycle. These species experience significant salinity fluctuations, necessitating adjustments in their ion transport pathways and maintaining effective electron transfer during the shift.
Freshwater Organisms:
Freshwater organisms, including specific fish species, amphibians, and microbes, have adapted to flourish in low-salinity habitats. These species generally possess highly efficient ion transport mechanisms that enable them to sustain intracellular ion concentrations despite the low salinity of their environment. These conditions optimise their respiratory chains, ensuring unaffected ATP production.
Electron Transfer at Reduced Salinity: Investigative Findings
Recent experimental studies have elucidated the electron transport chain’s functioning in low-salinity settings. Researchers have employed diverse methodologies to assess the efficacy of electron transfer and ATP synthesis under varying salinity conditions, elucidating the mechanisms that enable organisms to acclimatise to salinity variations.
Principal Experimental Methodologies:
Oxygen Consumption Measurements: By monitoring oxygen consumption rates, researchers can evaluate Complex IV’s activity (cytochrome c oxidase) and determine the overall efficiency of the respiratory chain.
ATP Production Assays: These assays enable researchers to quantify the ATP generated by cells under varying salinity conditions, offering a direct assessment of respiratory efficiency.
Ion Transport Studies: By looking at how ions move through cell membranes, scientists can figure out how living things keep their ion balance when the salt level is low and what that means for electron transport. Cryo-EM analysis.
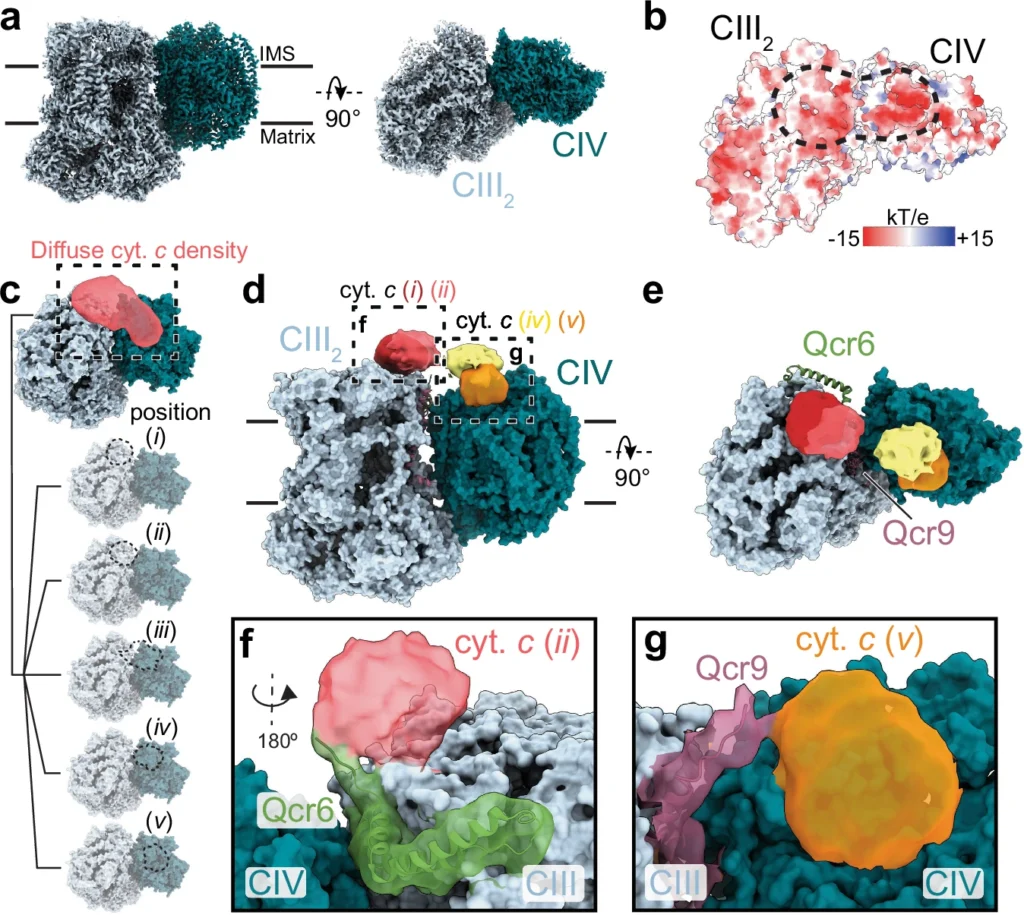
Applications of Biotechnology:
Understanding how salt influences electron transfer throughout the respiratory chain has significant implications for biotechnology. By using the understanding of organismal adaptation to low salinity, scientists can create bioengineered organisms with improved salinity tolerance for several uses.
Bioengineering for Improved Salinity Resistance:
The creation of bioengineered organisms capable of flourishing in low salinity habitats is a possible application. Industries like aquaculture, where variable salt levels can affect the health and production of cultivated species, may utilise these organisms. Enhancing these organisms’ salt tolerance may increase their development rates and overall health.
Applications in Energy Generation:
We may also use bioengineered organisms that exhibit improved respiratory chain efficiency in low-salinity settings for energy production. We could engineer microorganisms capable of effectively converting organic matter into biofuels in low-salinity circumstances for use in wastewater treatment facilities or similar environments.
Prospective Research Avenues:
Despite considerable advancements in comprehending electron transmission at low salinity, some concerns remain unresolved. Future research should focus on the following areas:
Looking into the Role of Specific Ions: More research is needed to find out exactly what role certain ions, like sodium and potassium, play in keeping the respiratory chain working in low-salinity conditions.
Adaptive Mechanisms: Further investigation is required to elucidate and define the molecular mechanisms enabling organisms to acclimatise to low-salinity settings.
Diverse Ecosystems: Expanding studies to include organisms from diverse habitats, such as extremophiles inhabiting hypersaline or brackish environments, may provide novel insights into the boundaries of electron transfer under extreme conditions.
Final Assessment:
Electron transfer within the respiratory chain is an essential mechanism that facilitates ATP synthesis in cells. Reduced salinity presents distinct obstacles to this process by disturbing ion equilibria, diminishing the proton gradient, and undermining protein stability. Many organisms have developed exceptional adaptations that enable them to sustain efficient electron transport and ATP synthesis, even in adverse situations. Comprehending these pathways not only augments our understanding of cellular respiration but also unveils new opportunities for biotechnological applications in fields such as aquaculture, energy generation, and environmental stewardship.
Frequently Asked Questions:
1). How does salinity affect the electron transport chain?
Salinity changes the balance of ions inside cells, which is important for keeping the electrochemical gradients that make electron transfer easier in the respiratory chain.
Can low salinity cause cellular damage?
2). Can low salinity lead to cellular damage?
Indeed, low salinity can disturb the ionic equilibrium and osmotic pressure, resulting in cellular swelling or shrinkage, which may compromise cellular integrity and hinder electron transmission.
3). In what manner do organisms acclimatise to situations with low salinity?
Organisms acclimate to low-salinity habitats through specialized ion transport systems, the synthesis of suitable solutes, and membrane modifications that maintain the respiratory chain’s functionality.
4). Under low salinity conditions, what function do ions serve in the electron transport chain?
Sodium and potassium ions are vital for sustaining membrane potential and the proton gradient, both of which are crucial for electron transport and ATP synthesis.
5). What are the possible applications of comprehending electron transport in low salinity conditions?
Biotechnology can use our understanding of electron transfer at low salinity to create bioengineered organisms for energy generation, aquaculture, and environmental remediation in areas with fluctuating salinity.
For more chemistry blogs, visit chemistry Master